Embrittlement Mechanisms and Material Effects
Hydrogen embrittlement refers to the loss of ductility and tensile strength of a steel due to the diffusion of atomic hydrogen into the metal’s crystalline structure. This hydrogen-induced degradation, also known as hydrogen stress cracking or delayed fracture, has resulted in catastrophic material failures across industries. Understanding the mechanisms of hydrogen embrittlement is critical for the safe design of high-strength steels and mitigation of damage risks.
Key Mechanisms
Three primary mechanisms are associated with hydrogen embrittlement in steels:
- Hydride-induced embrittlement: Hydrogen forms brittle hydride phases that nucleate and grow in regions of high stress and reduce toughness. This mechanism mainly affects hydride-forming alloys.
- Hydrogen-enhanced decohesion (HEDE): Accumulation of hydrogen atoms at defects lowers interatomic bond forces, promoting premature brittle intergranular or transgranular fracture. Hydrogen uptake increases with stress.
- Hydrogen-enhanced localized plasticity (HELP): Hydrogen accumulation at areas of high stress concentration enhances dislocation mobility and localized sliding between atomic planes, leading to microvoid formation and microcracking. Extensive plastic deformation occurs before macroscale fracture.

Susceptibility Factors
The risk and severity of hydrogen embrittlement depend on many factors:
- Alloy composition: Austenitic stainless steels more resistant than martensitic and ferritic steels. Rare earth elements may provide hydrogen trapping.
- Strength level: Higher strength steels more susceptible. Above 39 HRC hardness at greater risk.
- Grain structure: Finer grains and high dislocation density increase hydrogen diffusion rates.
- Stress level: Higher stresses promote hydrogen migration to crack tips and defects, accelerating embrittlement. Residual stresses also contribute.
- Exposure conditions: Pressure, temperature, time. Electroplating and corrosion environments provide hydrogen.
Material Effects
Key effects of hydrogen uptake on mechanical properties:
- Loss of ductility and toughness
- Increased crack growth rates
- Lowered tensile and fatigue strength
- Delayed brittle fracture after hydrogen exposure under stress
Test Methods for Hydrogen Embrittlement
Several standard test procedures characterize the susceptibility of steels to hydrogen embrittlement damage:
Electrochemical Hydrogen Charging
Electrolytically introduces diffusible atomic hydrogen into the steel microstructure under controlled conditions. Changes in mechanical properties before and after charging assessed.
Slow Strain Rate Testing
Measures loss of ductility during slow tensile testing in hydrogen-containing environments versus inert environments. Indicates sensitivity to hydrogen stress cracking.
Hydrogen Microprint Technique
Uses compressed hydrogen gas to create microscopic blisters/cracks on a steel surface. Size, morphology, and number provide a hydrogen damage susceptibility index.
Fracture Mechanics Testing
Determines crack growth rates and fracture toughness (KIC) in high-pressure hydrogen gas. Evaluates hydrogen effects on crack propagation.
Hydrogen Permeation
Measures the rate of hydrogen diffusion through a steel membrane. Provides hydrogen diffusivity and solubility data. Devanathan-Stachurski cell commonly used.
Preventing Hydrogen Embrittlement Damage
Recommended measures to avoid hydrogen embrittlement failure:
- Select less susceptible alloys, microstructures, strength levels
- Minimize residual and service stresses
- Use lower hydrogen processes like plasma/laser hardening
- Bake after plating to drive out absorbed hydrogen
- Apply inhibiting surface treatments
- Perform hydrogen embrittlement testing
- Improve design to avoid stress concentrations
Careful material selection and processing are required to prevent embrittlement, especially for high-strength steel components exposed to hydrogen.
The Complex Case of Hydrogen Embrittlement in Iron Aluminides
Iron aluminide alloys based on Fe3Al and FeAl are intermetallics under development for elevated temperature applications. However, their susceptibility to hydrogen embrittlement remains a key challenge limiting room temperature ductility and service in hydrogen-containing environments.
Unique Factors in Iron Aluminides
Several characteristics contribute to hydrogen embrittlement susceptibility:
- Ordered crystal structure: Favours hydrogen diffusion along anti-phase boundaries
- High strength: Increases hydrogen-stress interactions
- Grain boundaries: Provide hydrogen trapping sites
- Brittle intermetallic bonds: Prone to hydrogen-induced decohesion
Need for Fundamental Studies
Despite much research, the mechanisms enabling the severe hydrogen embrittlement in iron aluminides at room temperature are unclear. Most studies have focused on empirical property evaluation rather than deep understanding.
More rigorous fundamental investigations combining experiments, modelling, and simulations at the nanoscale are required to reveal the underlying hydrogen-microstructure interactions. Advanced microscopy and spectroscopy techniques are now available to probe these hydrogen effects and interactions directly. Progress in this area can enable the design of embrittlement-resistant iron aluminides.
In summary, hydrogen embrittlement remains a persistent challenge for steels, especially high-strength alloys used in hydrogen service environments. Both empirical studies and fundamental understanding of hydrogen-deformation interactions are needed to guide alloy and process improvements for damage resistance. Novel characterization methods provide pathways for deeper insight into hydrogen embrittlement mechanisms.
Hydrogen Embrittlement in Steel Pipes
Steel pipelines are critical infrastructure for distributing natural gas, oil, and other industrial fluids. However, the threat of hydrogen embrittlement limits the use of standard carbon and low alloy steel pipes for emerging applications like hydrogen transport. This article provides an overview of hydrogen embrittlement susceptibility factors for steel pipes and strategies to design embrittlement-resistant pipelines.
The Challenge of Hydrogen Gas Transport
Hydrogen gas is viewed as an important future energy carrier. However, hydrogen can diffuse into and embrittle conventional steel pipelines. Factors affecting susceptibility include:
Steel composition – High-strength microstructures like tempered martensite are more prone to embrittlement. Austenitic and duplex stainless steels provide greater resistance.
Operating pressures – Higher pressures increase hydrogen permeation and solubility, accelerating diffusion and embrittlement.
Cyclic stresses – Fluctuating pressure cycles enhance hydrogen transport and trapping at microstructural defects.
Residence time – Prolonged hydrogen exposure provides time for diffusion into the steel wall and accumulation at internal sites.
Environment – Contaminants like H2S increase susceptibility due to synergy effects.
Preventing Hydrogen Cracking Failures
Methods to design steel pipelines resistant to high-pressure hydrogen include:
- Specify maximum hardness – Keep strength below 950 MPa and hardness under 25 HRC
- Choose microalloyed or bainitic linepipe steels – Avoid hardened microstructures
- Use stainless steels like austenitic (300-series) or duplex alloys
- Apply inhibitors and coatings that mitigate hydrogen absorption
- Limit operating pressures and impose safety factors on design strength values
- Perform fatigue testing in high-pressure hydrogen to qualify materials
- Implement comprehensive inspection and monitoring programs to detect cracking
The safe design of steel pipes for pressurized hydrogen service requires selecting an intrinsically resistant alloy and microstructure, limiting stresses, and providing barriers to hydrogen ingress. Conservation measures and damage-tolerant operations are also key given the catastrophic nature of embrittlement failures.
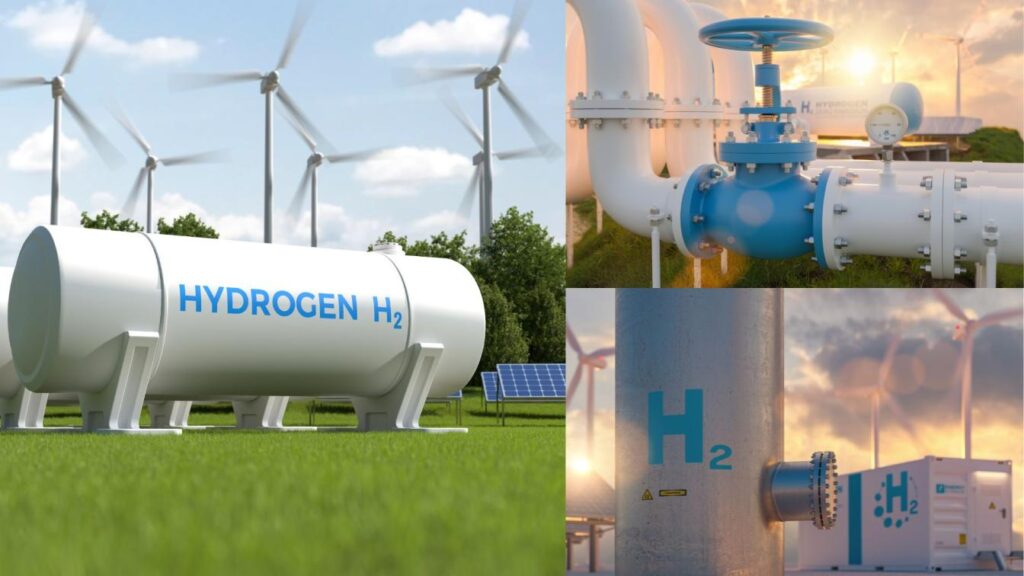
Mechanisms of Hydrogen Embrittlement in Steels
Hydrogen embrittlement refers to the degradation of the mechanical properties of steels due to the ingress and diffusion of hydrogen atoms into the metal’s crystalline structure. This phenomenon can lead to catastrophic and unexpected failures in steels exposed to hydrogen gas or aqueous environments. Understanding the mechanisms driving hydrogen embrittlement is key to mitigating damage and failures in steel components and infrastructure.
The Problem of Hydrogen Embrittlement
Several characteristics of hydrogen contribute to its potency in embrittling steels:
- Extremely small size enables diffusion through the crystal lattice
- Accumulation at microstructural defects and sites of stress concentration
- Reactions at interfaces weaken atomic bonds
- Buildup of internal hydrogen pressure
- Formation of brittle hydride phases
The result is degradation of the steel’s mechanical properties, including:
- Loss of ductility and toughness
- Increased crack propagation rates
- Reduced tensile and fatigue strength
- Delayed sudden fracture after hydrogen exposure under stress
Hydrogen embrittlement has been responsible for many failures in high-strength steels, resulting in catastrophic damage and economic losses across industries.
Key Mechanisms of Hydrogen Embrittlement
The three primary mechanisms proposed to explain hydrogen embrittlement in steels are:
Hydride Formation
- Hydrogen reacts with alloying elements like titanium, vanadium, or niobium
- Brittle hydride phases form in regions of high hydrogen solubility and stress
- Hydrides act as stress concentrators and crack initiation sites
- Mainly affects certain classes of alloys prone to hydriding
Hydrogen-Enhanced Decohesion (HEDE)
- Hydrogen accumulates at microvoids, carbides, grain boundaries
- Local hydrogen buildup reduces interatomic bonding forces
- Facilitates crack propagation along intergranular or transgranular paths
- Directly enhances brittle fracture processes
Hydrogen-Enhanced Localized Plasticity (HELP)
- Hydrogen accumulates at high stress regions near crack tips
- Enhances dislocation mobility and promotes localized sliding
- Leads to microvoid formation and microcracking
- Extensive plastic deformation precedes macroscale fracture
While aspects of all three mechanisms may contribute, their relative importance likely depends on the specific steel and conditions. More research is needed on hydrogen-deformation interactions at the crack tip to clarify dominant mechanisms.
Examples of Hydrogen Embrittlement Failures
Some notable examples highlighting the impact of hydrogen embrittlement include:
- Pipeline fractures – High-pressure gas transmission pipelines often fail due to hydrogen cracking, resulting in costly repairs and unsafe releases.
- Aircraft engine failures – Jet turbine blades made from high-strength martensitic steels fracture due to hydrogen exposure during operation, causing engine breakdowns.
- Bolting failures – Electroplated high-strength steel fasteners used in mechanical assemblies can catastrophically fail days or weeks after installation due to hydrogen.
Mitigation and Prevention
Strategies to mitigate hydrogen embrittlement damage include:
- Selecting steel alloys and tempers resistant to hydrogen cracking
- Using lower hydrogen processes like plasma nitriding to avoid hydrogen uptake
- Minimizing applied and residual stresses during manufacturing and service
- Baking after plating to drive off absorbed hydrogen
- Shot peening to induce compressive stresses that deter cracking
- Applying surface inhibitors that retard hydrogen absorption
Careful design and material selection is key to prevent embrittlement failures in high-strength steels used in hydrogen service environments across industrial sectors. Further research can help refine alloys, processes, and safety factors to enable the safe use of steels where hydrogen is present.
Hydrogen Embrittlement Mechanisms in Austenitic Stainless Steels
Austenitic stainless steels demonstrate excellent resistance to hydrogen embrittlement compared to other high-strength steels due to their FCC crystal structure and alloying additions. However, hydrogen can still degrade the ductility and fracture toughness of these alloys under certain conditions through complex mechanistic interactions. This article provides an overview of hydrogen embrittlement susceptibility factors and mechanisms in austenitic stainless steel alloys.
Reduced Susceptibility of Austenitic Stainless Steels
Key factors contributing to the relatively high resistance of austenitic stainless steels like grades 304 and 316 include:
- Face-centered cubic (FCC) crystal structure has limited interstitial sites for hydrogen
- Alloying elements like chromium increase binding forces for hydrogen
- Stacking faults and twins in FCC structure trap hydrogen and limit diffusion
- Lower yield strength and higher ductility inhibit crack initiation
- Corrosion resistance prevents surface hydrogen absorption
However, hydrogen can still accumulate at lattice defects and interfaces at sufficiently high fugacities, resulting in some degradation of mechanical properties.
Mechanisms of Hydrogen Damage in Austenitic Alloys
Two mechanisms are most relevant for austenitic stainless steels:
Hydrogen-Enhanced Decohesion (HEDE)
- Hydrogen buildup at internal interfaces reduces local cohesion
- Leads to intergranular separation and microvoid formation under stress
- Often linked to sensitization or formation of brittle phases
Localized Hydrogen Plasticity
- Dislocation movement concentrates hydrogen in slip bands through strain transport
- Results in planar slip, shear localization, and microvoid formation
- Affected by stacking fault energy and degree of strain hardening
While austenitic steels are highly resistant to bulk hydrogen embrittlement, localized damage through HEDE and plastic instability modes can still occur, especially for sensitized material and components with high triaxial stresses.
Preventing Hydrogen Cracking in Austenitic Components
Recommended strategies include:
- Solution annealing to dissolve carbide phases and ensure a single FCC phase
- Reducing residual stresses through design changes and processing adjustments
- Specifying maximum hardness levels to avoid extensive strain hardening
- Shot peening to impart compressive surface stresses
- Using cold work levels below 5-10% to limit strain hardening and slip planarity
- Applying protective coatings or inhibitors to block hydrogen absorption
For critical austenitic steel components, rigorous qualification testing in hydrogen environments provides the assurance needed to reliably withstand embrittlement risks.
Hydrogen Damage Mitigation for Steel Welds
Welding operations generate atomic hydrogen that can be absorbed into the microstructure of high-strength steel weldments, resulting in hydrogen cracking along or near fused areas. This article summarizes contributing factors, failure modes, and mitigation strategies for preventing hydrogen embrittlement damage in steel welds and heat-affected zones.
Hydrogen Uptake During Welding
Sources of hydrogen input during steel welding include:
- Dissociation of atmospheric H2O vapor near the arc
- Decomposition of hydrocarbon contaminants on the surface
- Hydrogen pickup from moisture or hydrocarbons in the electrode or flux
- Reactions with the slag formers and arc gases used in the process
The high temperatures of the welding arc and weld pool promote the rapid diffusion and solubility of atomic hydrogen into the microstructure of steel welds and nearby base material.
Characteristic Weld Embrittlement Failures
Typical modes of weld cracking due to hydrogen include:
Hydrogen-induced cold cracking
- Through-thickness cracking along the weld immediately after solidification
- Due to residual stresses and high local hydrogen concentrations
Delayed hydrogen cracking
- Fracture of low-toughness heat-affected zones days after welding
- Failures often triggered during post-weld stress relief heat treatments
Underbead cracking
- Longitudinal cracking confined to the region under the weld deposits
- Attributed to high hydrogen levels interacting with stress concentrations
Failures degrade the structural integrity and require extensive repairs.
Preventing Hydrogen Weld Cracking
Methods used to avoid hydrogen weld embrittlement include:
- Selecting low-hydrogen electrodes and filler material
- Using low-moisture fluxes and covering fluxes to exclude humidity
- Preheating steel above 200°F prior to welding to drive off moisture
- Post-weld baking between 1100-1250°F to diffuse out residual hydrogen
- Peening or shot blasting to impart compressive stresses that oppose cracking
- Specifying maximum hardness for base and weld metals
- Applying tempering or stress reliefs soon after welding when needed
For critical steel weldments, testing weld procedures and measuring diffusible hydrogen provides assurance of hydrogen embrittlement resistance.